Brain cells function using rapid electrical impulses, a process that underlies our thoughts, behavior, and perception of the world. Yet, for a long time, it's been challenging for scientists to see exactly how individual neurons work together in larger circuits.
Now, a new technique reported in Nature finally gives the clearest picture ever of brain cell activity. Using a voltage-sensing molecule that fluorescently lights up when brain cells are electrically active, researchers at Boston University and the Massachusetts Institute of Technology have shown that they can see the activity of many more individual neurons than before as they fire inside the brains of mice.
With the new voltage sensor, it is also possible to measure very small fluctuations in activity that occur even when a neuron is not firing a big spike in electrical activity. This could help neuroscientists study how small fluctuations impact a neuron's overall behavior, which has previously been very difficult to do in living brains, says BU biomedical engineer Xue Han, co-corresponding author on the paper, describing the advance.
This technique can be performed using a simple light microscope, and it could allow neuroscientists to link firing of certain cell groups to specific behaviors, says co-corresponding author Edward Boyden of MIT. "If you want to study a behavior, or a disease, you need to image the activity of populations of neurons because they work together in a network."
Until now, it's been possible to measure the electrical activity of neurons by inserting an electrode into the brain, but this technique is labor-intensive and typically only allows researchers to record activity from one neuron at a time. Multielectrode arrays can allow monitoring of electrical activity from many neurons at once, but it's impossible to record the densely packed activities of all neurons within a piece of brain tissue. A technique called calcium imaging does allow such dense sampling, but it measures calcium, which is an indirect and slow measure of neural electrical activity.
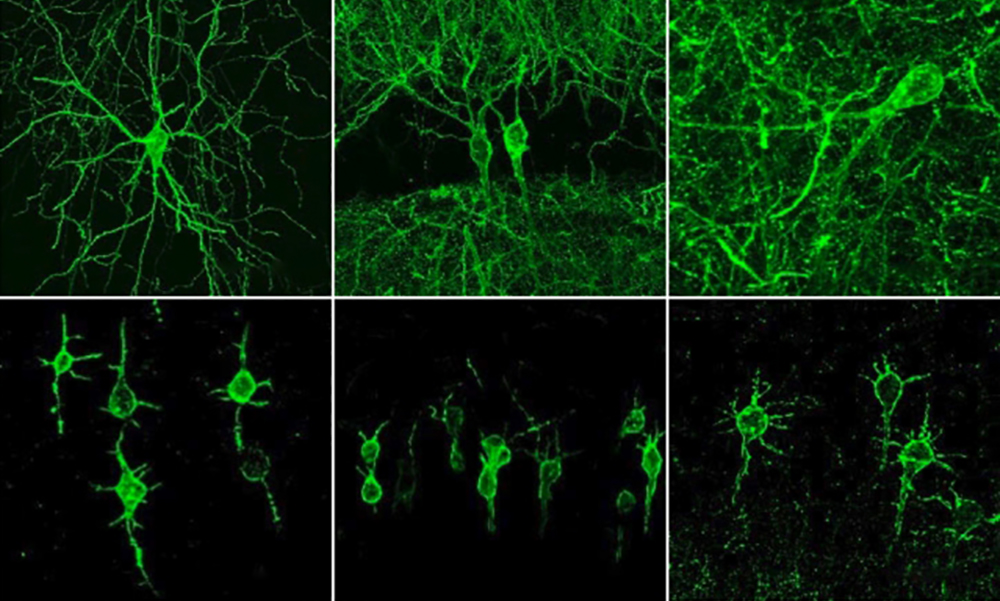
In 2018, Boyden's team first developed an alternative way to monitor electrical activity by labeling neurons with a fluorescent probe. They engineered a molecule called Archon1 that can be genetically inserted into neurons, where it becomes embedded in the cell membrane. When a neuron's electrical activity increases, the molecule becomes brighter, and this fluorescence can be seen with a standard light microscope. The researchers showed that they could use the molecule to image electrical activity in the brains of transparent worms and zebrafish embryos, and also in mouse brain slices. But for the new study, they wanted to try to use it in living, awake mice as they engaged in a specific behavior.
To do that, the researchers needed to modify the probe so that it would go to a more specific region of the neuron's cell membrane, because when the molecule inserts itself throughout the entire cell membrane, the resulting images are blurry from the sprawling tendrils-called axons and dendrites-that extend outward from neurons like the arms of a starfish. The research team engineered a new version of Archon1 that sticks specifically to membranes of the central cell bodies of neurons, but not its outer axons or dendrites. They named this modified voltage-tracking molecule SomArchon.
"With SomArchon, you can see each cell as a distinct sphere," Boyden says. "Rather than having one cell's light blurring all its neighbors, each cell can speak by itself loudly and clearly, uncontaminated by its neighbors."
To test how it worked in live mice, Boyden partnered with Han's lab at BU. "My lab has been doing a lot of work on imaging single neurons inside the brain," says Han, a BU College of Engineering associate professor of biomedical engineering. "We've used calcium imaging to look at a large number of neurons…but the timescale is very slow for calcium imaging. Voltage changes are all happening at the timescale of milliseconds. That's why we have been trying to do voltage imaging."
Genetically engineering live mice to have SomArchon in their brain cells, the research team imaged electrical activity in a part of the brain involved in planning movement, called the striatum, while the mice ran on a ball. They were able to monitor electrical activity in many neurons simultaneously and correlate each one's activity with the mice's movement. Some neurons' activity went up when the mice were running, some went down, and others showed no significant change. Former BU graduate researcher Seth Bensussen, one of three co-first authors on the study, led the team's brain imaging efforts, and BU research scientist Hua-an Tseng led the computational aspect of the work.
"Over the years, my lab has tried many different versions of voltage sensors, and none of them have worked in living mammalian brains until this one," Han says. "With this technology, we can look at subtle changes in how voltage travels through brain cell membranes. A big part of my research is looking at the dynamics related to Parkinson's disease and deep brain stimulation. These voltage sensors will allow us to study how individual neurons communicate to generate larger neurological phenomena. Ultimately, it could guide in the design of new therapies to treat neurodegenerative diseases."
The team also showed that the voltage sensors can be combined with optogenetics, a technique that Han and Boyden pioneered over a decade ago, that allows researchers to turn brain cells on and off with laser light. In this case, the researchers activated certain neurons with light and then measured the resulting electrical activity in these neurons.
This work was supported by funding from the National Institutes of Health, the National Science Foundation, the Howard Hughes Medical Institute Simons Faculty Scholars Program, the Human Frontier Science Program, the US Army Research Office, the Grainger Foundation, the Pew Foundation, and Boston University Biomedical Engineering Department.
This story was adapted from an MIT article originally written by Anne Trafton.