We are all familiar with elastic materials - just think of a rubber band which can return to its original shape after being stretched.
Authors
- Jack K. Clegg
Professor of Inorganic Chemistry, The University of Queensland
- Ben Powell
Professor of Theoretical Physics, The University of Queensland
- John McMurtrie
Professor of Inorganic Chemistry, Queensland University of Technology
Humans have used elastic materials for millennia. These days, they're in everything from optical fibres to aeroplanes and buildings. But until now, scientists haven't been able to pinpoint exactly how these materials return to their original shape. What happens at the level of their molecules?
Published today in the journal Nature Materials , our new study uses the properties of flexible crystals to understand how interactions between molecules give rise to elasticity. This provides new insight into the model of elasticity developed by English polymath Robert Hooke more than 300 years ago.
Our findings will allow us to develop new ways of designing components for complicated aerospace and building materials or electronic devices.
The mystery of elasticity
A material is elastic if it can return to its original structure after being deformed. For example, a rubber band goes back to its original shape after it's been stretched. However, it will snap if pulled too hard. This is known as a "non-elastic change" - it means the material can no longer return to its original shape.
The most useful elastic materials can undergo large changes in their structures and still return to their original shape. There are many engineering uses for this. As one example, bridges are designed to move elastically in high winds to prevent them from falling down.
All materials are at least a little bit elastic: they can restore themselves after very small changes in structure. If you shake a piece of paper, it will still lie flat. But if you fold it, the crease is permanent - a non-elastic behaviour that is essential for origami.
Prior to our research, there were two main approaches to understanding elasticity.
In the 17th century, Robert Hooke first described how elastic materials work . He discovered that the force needed to stretch an elastic material is proportional to the distance it is stretched, and described this mathematically.
However, knowing this doesn't provide much insight for chemists and physicists like ourselves, who work to develop new materials with better elastic properties.
More recently, computers have been used to calculate the elastic properties of a material using its structure and the basic laws of physics. But while it's nice for a computer to understand the problem, it doesn't necessarily make it easier for humans to grasp. This is where our work on flexible crystals comes in.
How can a crystal be flexible?
Crystals, which are normally hard and brittle, are made up of a repeating pattern of atoms or molecules. Because the atoms or molecules are stacked neatly in place, it is hard to move them.
This is why diamond - a crystal of carbon atoms - is hard, while coal, also mostly made of carbon but not a crystal, is soft and crumbly.
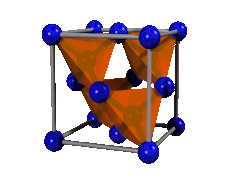
In the flexible crystals we have developed, there are weak interactions between the molecules. These crystals are made of a combination of simple organic molecules and metal ions.
Interactions between them allow the crystals to be bent so much, they can be tied in a knot without the crystal breaking.
Our new approach allows humans to understand how the subtle interactions between molecules in crystals give rise to elasticity.
We first used X-ray diffraction, a technique for determining the positions of atoms and molecules in crystals, at the Australian Synchrotron. This allowed us to understand how the arrangement of molecules in our flexible crystal changes when it's bent.
We then used a computer to model the interactions between pairs of molecules. Our results showed these interactions could be used to calculate elasticity just as accurately as theoretical models of the entire crystal.
So, what makes our crystal highly elastic? Our results show that none of the interactions between atoms are "happy" with the structure of the crystal when it is bent. Some would like it to move one way, others in the opposite direction. They have to compromise.
This means the molecules and atoms don't strongly resist to changes, making the crystal highly elastic despite its molecular structure which is typical of a regular, inflexible crystal.
We could not have learned this with either of the traditional approaches for analysing elasticity.
We were also able to calculate how much energy is stored within a crystal when it is bent, and found it was enough for the crystal to lift a mass 30 times its own weight one metre in the air. This is similar to shooting an arrow with a bow. When you draw the bow, you store elastic energy. Upon the release of the arrow, that elastic energy is transformed into kinetic energy - movement.
Our flexible crystals are not yet robust enough to be used in the construction of bridges or skyscrapers.
But the new understanding our study brings to elasticity could lead to new ways of preparing smart devices, wearable electronics, or even components for spacecraft.
Jack K. Clegg receives funding from the Australian Research Council
Ben Powell receives funding from the Australian Research Council and the Queensland Government.
John McMurtrie receives funding from the Australian Research Council.