Many of the biological materials that researchers are most interested in studying, including those associated with major diseases, don't lend themselves to the conventional methods that researchers typically use to probe a material's structure and chemistry.
One technique, called magic-angle spinning nuclear magnetic resonance, or MAS-NMR, has proven highly successful as a way of determining the properties of complex molecules such as some proteins. But the resolution achievable with such systems depends on the spinning frequency of tiny rotors, and these systems have bumped up against limits imposed by the rotor materials.
Most such devices used today rely on rotors made of yttria-stabilized zirconia, which are as thin as a pin. Such rotors fall apart if spun much faster than a few million revolutions per minute, limiting the materials that can be studied with such systems. But now, researchers at MIT have developed a method for making these tiny, precise rotors out of pure diamond crystal, whose much greater strength could allow it to spin at far higher frequencies. The advance opens the door to studying a wide variety of important molecules, including those found in the amyloid plaques associated with Alzheimer's disease.
The new method is described in the Journal of Magnetic Resonance, in a paper by MIT graduate students Natalie Golota, Zachary Fredin, Daniel Banks, and David Preiss; professors Robert Griffin, Neil Gershenfeld, and Keith Nelson; and seven others at MIT.
The MAS-NMR technique, Gershenfeld says, "is the tool of choice for [analyzing] complex biological proteins in biologically meaningful environments." For example, a sample could be analyzed in a liquid environment as opposed to being dried out or crystallized or coated for examination. "Only [solid-state] NMR does it in the ambient chemical environment," he says.
The basic method has existed for decades, Griffin explains, and involves placing a tiny cylinder filled with the material to be studied into a magnetic field where it can be suspended and spun up to high frequencies using jets of gas, usually nitrogen, and then zapped with radio-frequency pulses to determine key properties of the material. The term "magic angle" refers to the fact that if the cylinder containing the sample spins at one precise angle (54.74 degrees) relative to the applied magnetic field, various sources of broadening of the spectral lines are attenuated and a much higher-resolution spectrum is possible.
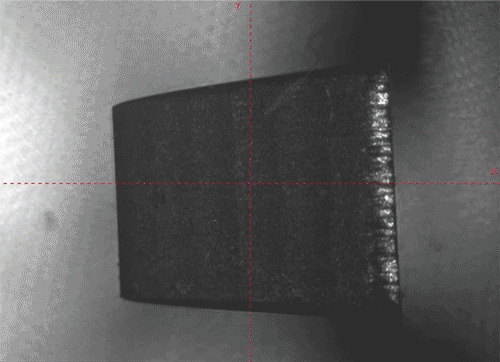
Courtesy of the researchers
But the resolution of these spectra is directly limited by how fast the tiny cylinders, or rotors, can spin before they shatter. Over the years, early versions were made of various plastics, then later ceramic materials were used, and finally zirconium, "which is the material of choice that most rotors are made of these days," Griffin says.
Such MAS-NMR systems are widely used in biochemical research as a tool for studying the molecular structure, down to the level of individual atoms, of materials including proteins that are difficult or impossible to probe using other standard lab methods. These include not only amyloid fibrils, but membrane proteins and some viral assemblies. But some of the most pressing challenges in both biomedical and materials science lie just beyond reach of the resolution of today's MAS-NMR systems.
"As we progressed to spinning frequencies above 100 kilohertz," equivalent to 6 million revolutions per minute, Griffin says, "these rotors have become very problematic. They fail about 50 percent of the time - and you lose a sample, and it destroys the NMR coil." The team decided to tackle the problem, which many said at the time was impossible, of making the rotors out of single crystal diamond.
Even the company making the laser system they used thought it couldn't be done, and it took years of work by an interdisciplinary team, involving students and researchers at both MIT's Center for Bits and Atoms and the Department of Chemistry, to solve that fabrication problem. (The collaboration grew out of Griffin and Gershenfeld serving on MIT's Killian Award Committee). They developed a kind of laser-based lathe system that rapidly spins a piece of diamond while zapping it with the laser, essentially vaporizing its outer layers until a perfectly smooth cylinder remains, just 0.7 millimeters across (about 1/36 inch). Then, the same laser is used to drill a perfectly centered hole through the middle of the cylinder, leaving a sort of drinking-straw shape.
"It's not obvious it would work," Gershenfeld says, "but the laser turns the diamond into graphite and drives the carbon off, and you can incrementally do that to drill deep into the diamond."
The diamond emerges from the machining process with a black coating of pure graphite, but the MIT researchers found that this could be eliminated by heating the rotor overnight at about 600 degrees Celsius (about 1,100 degrees Fahrenheit.) The result is a rotor that can already spin at 6 million revolutions per minute, the speed of the best zirconia rotors, and has other advantageous characteristics as well, including extremely high thermal conductivity and radio-frequency transparency.
Fredin points out that all the parts needed to make this high-precision machining system "were all designed and fabricated right here" in a basement lab in the Center for Bits and Atoms. "To be able to physically design and make everything and iterate it many times a day in-house was a crucial aspect of this project, as opposed to having to send things out to outside machine shops."
Achieving much higher spinning frequencies should now be possible with these new rotors, the researchers say, but will require the development of new bearings and new systems based on helium rather than nitrogen to drive the rotation, in order to achieve the increased speeds and the corresponding leap in resolution. "It was never worth it to develop these helium-compatible bearings for these small rotors until this technology was proven out, when the rotors previously used would not be able to withstand the spinning speeds," which could end up going as high as 20 million revolutions per minute, Golota says.
Such high rotation rates are almost unheard of outside the NMR field. Preiss says that as a mechanical engineer, "it's rare that you'd encounter something spinning above tens of thousands of rpm." When he first heard the 6 million rpm figure for these devices, he says, "I kind of thought it was a joke."
Because of these high speeds, Gershenfeld says, instabilities can easily arise from any imperfection: "If there's even a slight asymmetry in the structure, at these frequencies, you're doomed."
Golota says that in her experiments using current zirconia rotors, "when the rotors fail, they explode, and you essentially just recover dust. But when the diamond rotors fail, we were able to recover them intact. So, you're saving the sample as well, which can be an invaluable resource to the user."
They have already used the new diamond rotor to produce the carbon-13 and nitrogen-15 spectra of a small peptide, clearly demonstrating the capabilities of the new diamond rotor material, which Griffin says is the first new material for such rotors to be developed in the last three decades. "We've used spectra like these extensively," he says, "to determine the structure of amyloid-beta 1-42, which is a toxic species in Alzheimer's disease." Samples of such material are hard to get and usually obtainable only in tiny quantities, he says. "We now have a small rotor that's going to be hopefully very reliable where you can put in two or three milligrams of material and get spectral data like these," he says, pointing to the sample data they obtained. "It's really exciting and it's going to open up a lot of new areas of research."
This work "is truly remarkable," says David Doty, president of Doty Scientific, a maker of NMR systems, who was not involved in this work. "It would have been very hard to find anyone outside this group who would have thought it possible to laser machine diamond rotors with the precision needed for fast-MAS, prior to actually seeing it work," he says.
Doty adds, "What they have demonstrated thus far … is nothing short of amazing. If the additional needed progress can be made, hundreds of NMR researchers will want these to help them get better data for the projects they are working on, from improving our understanding of some diseases and developing better drugs to developing advanced battery materials."
"This new technology has the potential to be a game-changer in the way we will carry out solid-state NMR experiments in the future, opening unprecedented experimental opportunities in terms of resolution and sensitivity," says Anne Lesage, adjunct director of the institute of analytical sciences at the Ecole Normale Superieure in Lyon, France, who also was not associated with this work.
The research team also included Salima Bahri, Daniel Banks, Prashant Patil, William Langford, Camron Blackburn, Erik Strand, Brian Michael, and Blake Dastrup, all at MIT. The work was supported by the U.S. National Institutes of Health, the CBA Consortia fund, the U.S. Department of Energy, and the U.S. National Science Foundation.